A small bacteriophage protein determines the hierarchy over co ... - Nature.com
Abstract
Bacillus thuringiensis serovar israelensis is the most widely used biopesticide against insects, including vectors of animal and human diseases. Among several extrachromosomal elements, this endospore-forming entomopathogen harbors two bacteriophages: a linear DNA replicon named GIL01 that does not integrate into the chromosome during lysogeny and a circular-jumbo prophage known as pBtic235. Here, we show that GIL01 hinders the induction of cohabiting prophage pBtic235. The GIL01-encoded small protein, gp7, which interacts with the host LexA repressor, is a global transcription regulator and represses the induction of pBtic235 after DNA damage to presumably allow GIL01 to multiply first. In a complex with host LexA in stressed cells, gp7 down-regulates the expression of more than 250 host and pBtic235 genes, many of which are involved in the cellular functions of genome maintenance, cell-wall transport, and membrane and protein stability. We show that gp7 homologs that are found exclusively in bacteriophages act in a similar fashion to enhance LexA's binding to DNA, while likely also affecting host gene expression. Our results provide evidence that GIL01 influences both its host and its co-resident bacteriophage.
Introduction
Most bacteria carry prophages. These are genomes of temperate bacteriophages that have critical roles in shaping bacterial ecology and in some cases enable the virulence of pathogens1,2,3. During infection, temperate phages can enter lysogeny (i.e., a dormant state within the bacterium) or activate the lytic cycle to produce new phage progeny. Temperate phages have evolved a range of mechanisms to promote these opposite lifestyles4,5, although, how these are controlled and how the phage takes over the host processes is only understood for a small number of phages.
To achieve stable lysogeny, some temperate phages rely on their own transcription repressors with embedded auto-proteolytic activity in the dimerization domain6,7,8. Upon DNA damage and SOS response induction, the active RecA filament (RecA*) that is formed on single-stranded DNA at sites of DNA damage triggers the self-cleavage and inactivation of the repressor; this drives the phage into the lytic cycle. In contrast, other phages encode for repressors where the DNA-binding activity is abolished by an anti-repressor protein4,9,10 or a dedicated protease5, both of which can be regulated through a variety of mechanisms. Other phages, such as tectivirus GIL01 that infects the spore-forming bacterium Bacillus thuringiensis serovar israelensis, a widely used biopesticide, do not encode their own repressor, but use the host LexA to establish the prophage quiescent state11.
The GIL01 15-kb linear genome does not integrate into the host chromosome and has terminal proteins covalently linked to each 5'-end. GIL01 replicates its genome via a terminal protein-primed amplification using its own DNA polymerase12. The genome of GIL01 includes 30 open reading frames (ORFs) divided into two main modules that are transcribed in the same direction13,14. The tandem promoters P1 and P2 are located upstream of the eight genes (ORF1-ORF8) that constitute the module for proteins involved in the regulation of the GIL01 genome's replication and transcription. The promoter P3 controls the expression of the downstream genes involved in the host recognition, the assembly of the capsid (which is composed of an outer protein layer and an inner protein-rich lipid membrane), and cell lysis15. In a previous study we established that the GIL01-encoded small proteins gp1 and gp7 are involved in lysogeny, and mutations in either of these proteins lead to the lysogenic state not being established14,16. Gp7 forms a complex with the host LexA repressor and acts as a scaffold to set the repressor in a conformation that is specific for binding to target DNA sites16,17. In doing so, gp7 acts as a co-repressor that subverts host LexA to occupy two operators at P1, one of which lacks a canonical LexA sequence motif. By expressing gp7, GIL01 uses LexA as a reliable indicator of the host's viability. In addition, gp7 has a dual action on RecA, by increasing LexA's affinity for the recA operator and by impairing the RecA-stimulated self-cleavage of LexA, which might alter the dynamics of the host SOS response16,17.
Serovars of B. thuringiensis carry a diverse pool of self-replicating extrachromosomal DNA molecules. For instance, nine plasmids have been described for serovar israelensis, which include the well-characterized pBtoxis that encodes the entomopathogenic crystals produced during sporulation, and two prophages, i.e., the linear double-stranded DNA replicon GIL01 and the circular prophage pBtic23511. pBtic235 is a 235-kb low-copy-number element that is composed of plasmid and phage modules that are mainly encoded on the sense and anti-sense strands, respectively11. pBtic235 carries 287 genes and appears to influence the host bacterium's lifestyle, as it encodes predicted homologs of metabolite transporters, tellurium resistance, SOS-inducible phage functions, and stress-response and genome-maintenance functions, such as uvrD helicase. In addition, pBtic235 encodes its own transcription factors, such as sigma-70 factor, several tRNA molecules, and determinants involved in genome replication, which combined with a large genome size, are a feature of jumbo phages18. This extrachromosomal element appears to transiently integrate its genome into the host chromosome, where it is believed to have a role in terminating the chromosome's replication19. The potential molecular cross-talk between pBtic235 and GIL01 and its effects on the lifestyle of B. thuringiensis serovar israelensis remain unstudied. Here, we describe how the GIL01-encoded co-repressor gp7 coordinates the induction of prophage pBtic235 and affects the overall transcription in B. thuringiensis serovar israelensis. On the computational side we provide a pipeline for a reproducible and transparent RNA-sequencing analysis.
Results
B. thuringiensis serovar israelensis and prophage pBtic235 change transcriptional programs in response to prophage GIL01 induction
GIL01 establishes a lysogenic cycle in the infected B. thuringiensis serovar israelensis strain GBJ002, a 4Q7 derivative cured of all plasmids and three chromosomal prophages, except for the pBtic235 prophage (Supplementary Fig. 1)19. Our aim was to to study transcription in strain GBJ002 in presence of GIL01. To this end, we determined and compared the transcriptomes in the GIL01 and pBtic235 lysogenic strain GBJ002(GIL01) and its GIL01 cured derivative, GBJ002, treated or not with 100 ng mL−1 of the DNA-damaging agent mitomycin C (MMC). We allowed the strain to develop as far as the early exponential growth phase, after which the cultures were split in two and MMC was added to one half of the culture to induce the SOS response to DNA damage. Samples for RNA sequencing (RNA-seq) were taken 30 min after MMC addition, which corresponds to the time just before the burst of GIL01 virions20,21. Gene-expression profiles were then determined using differential expression analysis (see Methods).
In strain GBJ002, 26.7% (1166 chromosomal genes, 240 pBtic235 genes) of the genes showed changes in expression levels after treatment with MMC (Fig. 1a; Supplementary Data 1). As expected, there was an increase in expression of the core SOS-response genes lexA (2.6-fold), recA (4.6-fold), dinB (DNA polymerase IV, 4.9-fold), and uvrABC (exonuclease components, 2.4- to 11.3-fold), and of several other genes involved in the transposition or upkeep of genome integrity, such as a transposase (3.8-fold) and topoisomerase IV genes A and B (6.3-fold and 6.2-fold, respectively). Interestingly, 235 of the 287 (82%) differentially expressed pBtic235 genes were upregulated (Supplementary Data 1). Consistent with a previous report showing the induction of prophage pBtic235 after MMC treatment22, the upregulated genes included 68 genes encoded on the anti-sense strand of pBtic235, corresponding to the phage module including the phage capsid proteins and lytic genes, whereas the remaining 172 genes were encoded on the plasmid module. These included a RecA homolog (23.8-fold) that shares 42% amino acid identity with the host RecA, a LigA homolog (3.6-fold), a UvrD-helicase-like protein (46.9-fold), a crossover junction endodeoxyribonuclease RuvC (7.9-fold), 18 tRNA genes (10.9- to 61.8-fold), and a replication initiator protein A (RepA) (23.7-fold), indicating the involvement of pBtic235 in maintaining genome DNA integrity and replication of plasmid pBtic235 when the host is exposed to genotoxic conditions.
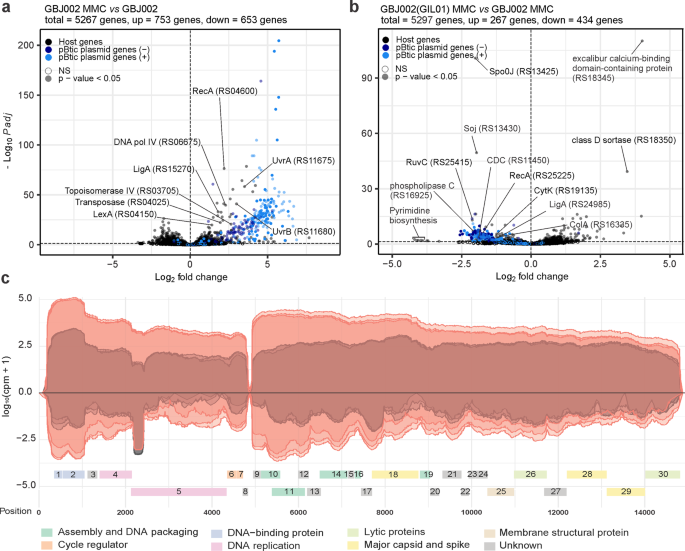
Volcano plots showing gene-expression changes between: a MMC-treated (100 ng mL−1, 30 min) and untreated GBJ002 cultures; b MMC-treated (100 ng mL−1, 30 min) GBJ002(GIL01) and GBJ002 cultures. The pBtic235 genes encoded on the sense (+, plasmid module) and anti-sense (−, phage module) strands, and the host genes are shown in the indicated colors. A significance cut-off of a FDR-adjusted p-value (Padj) of ≤0.01 was used. Genes without statistically significant fold-changes are indicated as NS. CDC, cholesterol-dependent anthrolysin O/cereolysin O family member protein and ColA, collagenase. Experiments were performed in three biological replicates. c GIL01 transcriptome in strain GBJ002(GIL01) 30 min after MMC treatment. The graph represents the GIL01 genome (ORF1-ORF30) with normalized read coverage for three biological replicates treated with MMC (100 ng mL−1) (read coverage in red) compared to their respective untreated samples (read coverage in gray). Reads are represented on a logarithmic scale (log10 counts per million). Gene features are colored based on putative or known gene functions15. Experiments were performed in three biological replicates.
To determine whether prophage GIL01 affects the host and pBtic235 transcription we performed a differential expression analysis between strains GBJ002(GIL01) and GBJ002. We found that GIL01 triggered extensive changes in the transcriptome of GBJ002(GIL01), as 7.4% of the 5267 genes were differentially expressed in non-induced cells and 12.7% in cells induced by DNA damage (Fig. 1b, Supplementary Data 2 and 3). The presence of GIL01 increased the expression of 237 and 123 and decreased the expression of 229 and 134 bacterial genes in the MMC-treated and untreated cells, respectively. In both cases most of the genes with increased expression had no annotated function or the genes could not be grouped according to gene ontology. In response to DNA damage, we detected the induction of all GIL01 prophage determinants (ORF1-ORF30) except for ORF8 (Supplementary Data 2).
Among the most down-regulated genes in presence of GIL01 prophage and induced DNA damage were the determinants involved in various functions, such as: pyrimidine biosynthesis (e.g., pyrB encoding aspartate carbamoyltransferase, pyrK and pyrD encoding subunits of dihydroorotate oxidase, pyrC encoding dihydroorotase, carB encoding a subunit of carbamoyl-phosphate synthetase; 18.4- to 9.9-fold), the transport of substrates through the cell wall (e.g., ABC transporter permeases, PTS transporter all without annotated gene names; 5.5- to 4.3-fold), virulence (e.g., M4 family metallopeptidase, plc encoding phospholipase C, cholesterol-dependent anthrolysin O/cereolysin O family member, nheA, nheB, and nheC encoding nonhemolytic enterotoxin, cytK encoding hemolysin IV; 4.3- to 2.0-fold) and sporulation (e.g. spo0J encoding stage 0 sporulation protein, soj encoding sporulation initiation inhibitor protein, 5.0- to 2.9-fold), among others (Fig. 1b, Supplementary Data 2). A similar profile of silenced genes was also observed in the GIL01 and pBtic235 lysogen, GBJ002(GIL01), which grew under non-stressed conditions, supporting lysogenic growth (Supplementary Data 3). Remarkably, the GIL01 prophage decreased the expression of more than 130 genes of the pBtic235 plasmid and phage modules under both conditions (Fig. 1b, Supplementary Data 2 and 3), suggesting that the GIL01 prophage inhibits the induction of the cohabiting pBtic235 prophage.
The transcriptional landscape of GIL01 confirmed the presence of two main transcription modules, which were both upregulated in response to the DNA damage (Fig. 1c). The upstream module encompassed genes encoding for gp1-gp8, which are responsible for genome replication and transcription regulation. Thirty minutes into the DNA-damage response (Supplementary Data 4), 55.9-fold more sequencing reads were assigned to this module compared to the untreated samples (Fig. 1c). Interestingly, in the absence of MMC, most reads assigned to the GIL01 anti-sense strand matched ~230 nucleotides near the 5'-end of the DNA polymerase gene (Fig. 1c). An analysis of the transcript in all three reading frames revealed no ORF with a length of >35 amino acids, which suggested that this region was non-coding. As this transcript levels decreased almost in proportion to the accumulation of transcripts for the two genes involved in the GIL01 genome replication in the DNA-damage response (i.e., gp4, gp5), this suggested an anti-sense interference mechanism of action. Further work is required to confirm the biological role of this highly abundant transcript.
According to our transcriptomic data the downstream 10-kb module of GIL01 encompasses genes 9 to 30, which encode the structural, lytic, and host recognition functions. Interestingly, both modules showed relatively high expression levels in the cells not treated with MMC. As the RNA-seq data in Fig. 1c represent the mean gene expression of the bacterial population, the apparent high expression of the GIL01 genes might reflect a high, spontaneous prophage induction within a portion of the population.
To confirm that spontaneous induction is indeed an intrinsic property of GIL0113, the number of plaque-forming units (PFUs) on the recipient host B. thuringiensis HER141023 was determined in a supernatant of GBJ002(GIL01) cultures treated or not with MMC during the exponential growth phase. These showed titers of approximately 107 and 1010 PFU mL−1, just before and one hour after the induction of the host SOS response (Table 1). It is worth noting that the pBtic235 virions, but not the GIL01 virions, are inactivated by vortexing the phage samples (Table 1). This allowed us to inactivate only phage pBtic235 in the samples. Our results indicate a high frequency of spontaneous induction of GIL01, which explains the high expression of the structural and lysis determinants of GIL01 in bacteria without chemical induction (Fig. 1c).
Gp7 regulates host gene expression and represses pBtic235 genes after DNA damage
In previous work we established that GIL01 protein gp7 is needed for lysogeny and exerts its co-repressor function by interacting with the host repressor LexA16,17. Here, we investigated the effects of gp7 on the host and pBtic235 transcriptional programs. For this we transformed strain GBJ002 with a vector for the IPTG-induced expression of gp7 [GBJ002(pDG7)] or its empty version [GBJ002(pDG)]. The strains were treated with MMC to induce the SOS response to DNA damage and prophage pBtic23522. RNA-seq and differential expression analysis were then used to profile the gene expression, both before and 1 h after the DNA damage. It is worth noting that quantitative PCR analysis (Supplementary Methods) shows that gp7 mRNA levels are comparable when gp7 is expressed from the IPTG-induced pDG7 plasmid or from the MMC-induced GIL01 prophage carried by the GBJ002(GIL01) strain (Supplementary Fig. 2). Differential expression analysis between the MMC-treated strains GBJ002(pDG7) and GBJ002(pDG) revealed substantial changes across the B. thuringiensis transcriptome, especially within the pBtic235 phage module. Gp7 affected the expression of 58 chromosomal genes and 226 pBtic235 genes (Fig. 2a; Supplementary Data 5). It predominantly down-regulated gene expression, repressing several host core SOS genes (e.g., recA, uvrAB, dinB, by ~2-fold) and genes involved in cell-wall transport and integrity, as well as in protein and nucleic acid hydrolysis (Supplementary Data 5). Interestingly, among the few genes that gp7 upregulated in the stressed cells there were several virulence factors24: the two pore-forming cytolysins hemolysin IV (CytK) by 2.3-fold, the cholesterol-dependent anthrolysin O/cereolysin O family member by 3.3-fold (GenBank ID, CP051858; locus tags, RS19135, RS11450, respectively), collagenase ColA by 2.5-fold, and phospholipase C by 2.3-fold (locus tags, RS16335, RS16925, respectively). This finding suggests that gp7 induced the virulent properties of B. thuringiensis during stress (Fig. 2a; Supplementary Data 5). However, the data contrast with the results showing that the GIL01 prophage down-regulates these virulence determinants (Fig. 1b, Supplementary Data 2), indicating that other unknown regulatory mechanisms are at play.
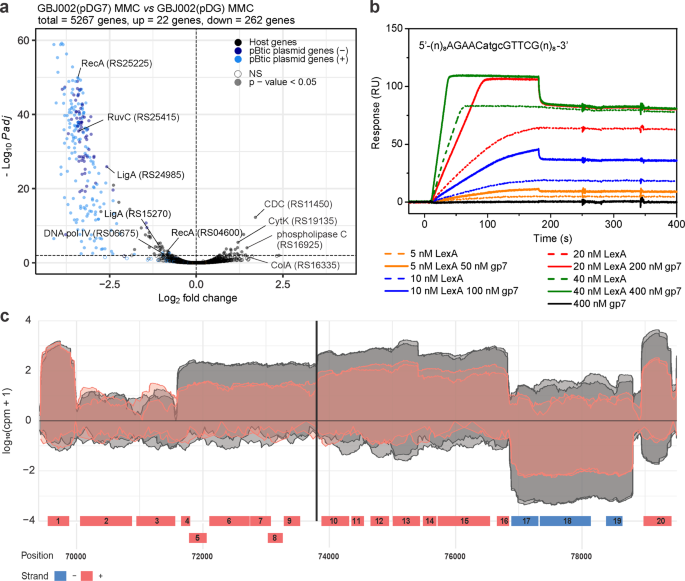
a Volcano plot showing the changes in gene expression between MMC-treated (100 ng mL−1, 1 h) and IPTG-induced (0.1 mM) GBJ002(pDG7) and GBJ002(pDG) cultures. The pBtic235 genes encoded on the sense (+, plasmid module) and anti-sense (−, phage module) strands, and the host genes are shown in the indicated colors. A significance cut-off of a FDR-adjusted p-value (Padj) of ≤0.01 was used. Genes without statistically significant fold-changes are indicated as NS. CDC, cholesterol-dependent anthrolysin O/cereolysin O family member protein and ColA, collagenase. Experiments were performed in two biological replicates. b Surface plasmon resonance sensorgrams of LexA (5-40 nM) interactions in the presence (solid lines) and absence (dotted lines) of gp7 (50–400 nM), with ~40 response units (RUs) of chip-immobilized LexA operator (sequence indicated above sensorgrams; pBtic235 coordinates 73794 to 73807) in pBtic235 (sequence above the diagram). Gp7 (400 nM) alone does not interact with the LexA operator (black line). Experiments were performed in three technical replicates and the represenatative sensorgrams are shown. c Read coverage (log10 counts per million) of the pBtic235 genomic region (from 70 to ~79 kb) flanking the LexA operator site (black vertical line) 1 h after MMC treatment (100 ng mL−1) in strain GBJ002(pDG7) with ectopically expressed gp7 (read coverage in red) and in strain GBJ002(pDG) without gp7 (read coverage in gray). The experiment was performed in two biological replicates. Genes labeled 1–20 correspond to the pBtic235 genes HIS92_RS25435 to HIS92_RS25530, respectively. Most lack functional annotation, except for the genes labeled 2 (HIS92_RS25440; phage repressor protein/anti-repressor Ant) 13 (HIS92_RS25495; nucleoside 2-deoxyribosyltransferase) 15 (HIS92_RS25505; metallophosphoesterase) and 18 (HIS92_RS25520; PD-(D/E)XK nuclease family protein).
A comparison of the pBtic235 transcriptome landscape in the MMC-treated GBJ002(pDG7) and GBJ002(pDG) strains shows that gp7 repressed gene expression of both the plasmid and the phage module of pBtic235 (Fig. 2a). For most differentially expressed pBtic235 genes, the functions were not annotated and genes of known functions are mostly involved in protein synthesis and maintenance of genome integrity (Supplementary Data 5).
We wanted to understand the molecular mechanisms by which gp7 coordinates the induction of pBtic235. First, we asked whether pBtic235 is regulated by LexA, and screened regions 300 bp upstream of pBtic235 genes for sequence motifs within promoter regions consistent with the LexA consensus motif of B. subtilis25 using xFITOM26. The search revealed a putative LexA binding sequence located between coordinates 73794 and 73807 in the pBtic235 sequence (GenBank ID, NZ_CP051859) (Fig. 2b, Supplementary Fig. 1). Next, we investigated the interaction of purified B. thuringiensis LexA with a DNA fragment carrying the putative LexA binding sequence identified using surface plasmon resonance (SPR) spectrometry. As we have previously shown that gp7 enhances LexA binding to the GIL01 genome and host SOS promoter sites16, we tested whether gp7 favored LexA binding to the putative target site on pBtic235. Our SPR data showed that LexA bound to the target site on pBtic235 and that gp7 enhanced LexA binding to the DNA, but did not interact directly with the LexA operator (Fig. 2b).
The identified LexA target site was located upstream of a putative operon that consist of seven ORFs (gene IDs, RS25480 to RS25510), one of which showed homology with the nucleoside 2-deoxyribosyltransferase (RS25495) and another with metallophosphoesterase (RS25505). The sequences of the remaining ORFs did not match any genes with known functions. A closer look at the transcriptomic data for GBJ002(pDG7) (Fig. 2a, Supplementary Data 5) revealed that ORFs RS25480 to RS25510 were repressed by gp7 (Fig. 2c). Together, the SPR spectrometry and transcriptomic data suggested that these ORFs were organized into an operon that is repressed by LexA and its co-repressor gp7.
In presence of gp7, we also observed the repression of genes RS25450 to RS25475 and RS25515 to RS25530 (Fig. 2c) despite the absence of a detectable LexA binding site upstream these genes or their presumed operons. It is therefore possible that ORFs RS25480 to RS25510 encode a regulator controlling the expression of those genes or that gp7 enables LexA binding to poorly conserved sites, as observed in phage GIL0116.
Gp7 represses the induction of the jumbo phage pBtic235
Our transcriptomic and SPR data raised the question whether gp7 coordinates the cross-talk between the transcriptional programs of GIL01 and pBtic235, to orchestrate the induction of both prophages in B. thuringiensis. To test this, we compared the growth dynamics of strain GBJ002(GIL01) and the pBtic235 lysogen cured of GIL01 (GBJ002), the latter with and without IPTG-induced gp7. First, we determined the level of spontaneous induction of the pBtic235 prophage by analyzing the formation of phage plaques on the strain HER141022. In the exponential growth phase of the GBJ002(pDG) strain, we detected a spontaneous induction of pBtic235 prophage of 7.5 ×104 PFU mL−1 (Table 1). At 4 h after the induction of the DNA damage, the number of pBtic235 infectious particles increased 240-fold. Next, we determined the extent of gp7 on pBtic235 virion production. Gp7 did not have detectable effects on cell growth under normal conditions, but after 4 h of inducing DNA damage, gp7 protected the cells from pBtic235-induced lysis (Fig. 3a), and we measured by up to 1000-fold reduction in pBtic235 plaque-forming units released in the medium of GBJ002 expressing gp7 (Fig. 3b, c and Table 1, compare GBJ002(pDG7) and GBJ002(pDG)). In contrast, the GBJ002(GIL01) strain carrying both the GIL01 and pBtic235 prophages, lysed at the fastest rate, as its culture was completely lysed 2 h after MMC treatment (Fig. 3a, d). Consistent with the observed down-regulation of pBtic235 prophage genes by the GIL01 prophage or by ectopically expressed gp7 (Figs. 1b and 2a), these data show that gp7 of phage GIL01 inhibits the production of pBtic235 virions under DNA-damaging conditions. To better investigate the role of LexA in the induction of prophage pBtic235, we used a GBJ002 derivative strain GBJ396 carrying a gene for LexA repressor that is incapable of self-cleavage14. We sequenced strains GBJ002 (SRA ID: SAMN30884992) and GBJ396 (SRA ID: SAMN30884991) and alignment of the sequencing reads revealed that the GBJ396 strain lacks the pBtic235 prophage. Using the soft agar overlay method with the GBJ396 strain as host, we observed that pBtic235 was unable to infect GBJ396.
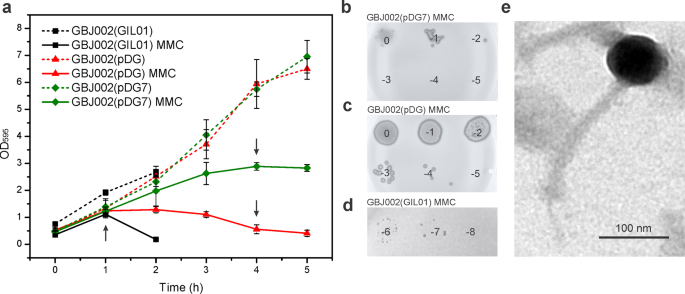
a Comparison of growth kinetics of B. thuringiensis strains GBJ002(GIL01) (black), GBJ002(pDG) (red), and GBJ002(pDG7) (green). IPTG (0.1 mM) was added to GBJ002(pDG) and GBJ002(pDG7) cultures after dilution of the overnight culture in fresh LB medium. MMC (100 ng mL−1) was added to half of the cultures in the early exponential phase (OD595, 0.3–0.4) at time zero. Bacterial density of the MMC-treated cultures is shown with solid lines, and that of the untreated cultures is shown with dotted lines. Bacterial growth was measured for 5 h in 1-h intervals or until complete cell lysis of MMC-treated GBJ002(pDG) culture. Black arrows indicate the times at which the culture samples were collected for determination of phage counts in b–d. Each data point represents the mean of three replicates. Error bars indicate standard deviations. Spot titers of MMC-treated culture supernatants of strains GBJ002(pDG7) b, GBJ002(pDG) c and GBJ002(GIL01) d on the recipient host, B. thuringiensis HER1410. The experiment was performed in duplicate. e Representative transmission electron micrograph of the pBtic235 phage showing an isometric head of ~80-nm diameter and an ~200-nm-long tail. Scale bar, 100 nm.
To further characterize the phage pBtic235 we induced its lytic cycle in strain GBJ002 with MMC, and generated pBtic235 plaques on the recipient strain. Although pBtic235 mostly formed turbid plaques, there was a single clear plaque, which suggested a lytic phage variant that could not establish the dormant state in the cell. We performed several rounds of propagation with the clear-plaque variant during repeated infections of HER1410, and extracted its DNA from the confluent plaques for sequencing. We mapped the reads to the published pBtic235 genome, which confirmed that the lytic plaques contained the pBtic235 genome22. The largest assembled contig sequence of 225.5 kb aligned with the deposited 235 kb pBtic235 sequence22 (GenBank ID, NZ_CP051859), with the exception of a single nucleotide change that resulted in an alanine-to-valine substitution of residue 925 of the minor structural protein carrying a carbohydrate-binding domain (NCBI Protein ID, AFQ30225). Remarkably, the read assembly revealed a ~10-kb deletion in the clear-plaque variant, which encompassed 12 genes (Supplementary Fig. 3), including repA, which indicated that pBtic235 genome replication can initiate without RepA. We detected multiple reads that connected the ends of the deleted region, showing that like the wild-type pBtic235 genome, the mutant pBtic235 genome was also circular19. Next, we purified the pBtic235 virions from the MMC-induced culture of GBJ002 and visualized their morphology using transmission electron microscopy. The micrographs showed that the phage pBtic235 belongs to the order Caudovirales, as it was composed of an isometric head of ~80-nm in diameter (edge-to-edge) and a tail of ~12 nm in width and ~200 nm in length, with a central tail fiber or spike at the base plate (Fig. 3e).
Tectivirus-borne gp7 homologs enhance the DNA-binding activity of LexA
Considering the global regulatory role of gp7 in B. thuringiensis we investigated the existence of gp7-like proteins in other bacteria. In a previous study we established that gp7 can interact with the LexA of Staphylococcus aureus and enhance its DNA binding17. Therefore, proteins with similar functional properties to gp7 might modulate the LexA activity in S. aureus. This might also be the case in Escherichia coli, where an unidentified factor appears to be required for LexA binding to multiple target sites27. These observations prompted us to search for LexA co-regulatory proteins in S. aureus and E. coli using affinity chromatography and mass spectrometry (Supplementary Methods), with S. aureus and E. coli LexA as bait, and the respective cleared cell extracts as the sources of potential gp7-like proteins (Supplementary Data 6). In addition, we applied the ProBiS algorithm28 to search the SWISS-MODEL Repository for proteins of E. coli, S. aureus, and B. thuringiensis that consist of 40–100 amino acids and share surface structural features with gp7. Using these approaches we were unable to identify a gp7-like protein (Supplementary Fig. 4).
We previously identified gp7 homologs exclusively in genomes of GIL01-related tectiviruses infecting Bacillus spp.29, although it is not known whether these have a similar role by interacting with LexA. Here, we purified six gp7 homologs from phages infecting insect, fish, and human pathogens Bacillus thuringiensis serovar aizawai, Bacillus mycoides, Bacillus anthracis, and Bacillus cereus (Supplementary Figs. 5 and 6). The sequence alignment of these gp7 homologs showed that they mainly differed in their C-terminal domain (Fig. 4a), which we previously found to be an unstructured region with at least the last six C-terminal residues not being required for the formation of the LexA complex17. To test whether the six gp7 homologs can form a complex with LexA we used SPR to measure the protein-protein interactions. B. thuringiensis LexA was immobilized on the surface of the SPR sensor chip, and gp7 or one of its homologs was injected over the chip. All six gp7 homologs interacted with the B. thuringiensis serovar israelensis LexA, and this interaction was concentration dependent, with KDs similar to that of gp7, in the range of 148 nM to 274 nM (Fig. 4b). It is of note that the LexA repressors of B. thuringiensis serovar aizawai or israelensis, B. mycoides, B. anthracis, and B. cereus share >96% identity (Supplementary Fig. 7). To test the activity range of the gp7-like proteins beyond Bacillus spp., we exposed the six homologs to S. aureus and E. coli LexA and found that all of these interacted with S. aureus LexA (KD from ~300–790 nM) (Supplementary Fig. 8), although they did not significantly interact with the E. coli LexA (Supplementary Fig. 9). The S. aureus and E. coli LexA share 67% and 38% identity, respectively, with the B. thuringiensis serovar israelensis LexA, which indicated that LexA-sequence conservation is needed for gp7 or its homologs to interact with LexA. As previously seen for gp7, no gp7-like protein interacted directly with the LexA operators from the GIL01 genome (Supplementary Fig. 10), and all of the homologs enhanced B. thuringiensis LexA binding to its cognate operator sequence. It is of note that of the three LexA repressors tested, the LexA proteins from S. aureus and B. thuringiensis showed the highest sequence identities17. Moreover, all the gp7 homologs tested enhanced the interaction of B. thuringiensis LexA with the cognate operator sequence (Fig. 4c) and we would expect them to co-regulate the DNA-damage responses in their respective hosts.
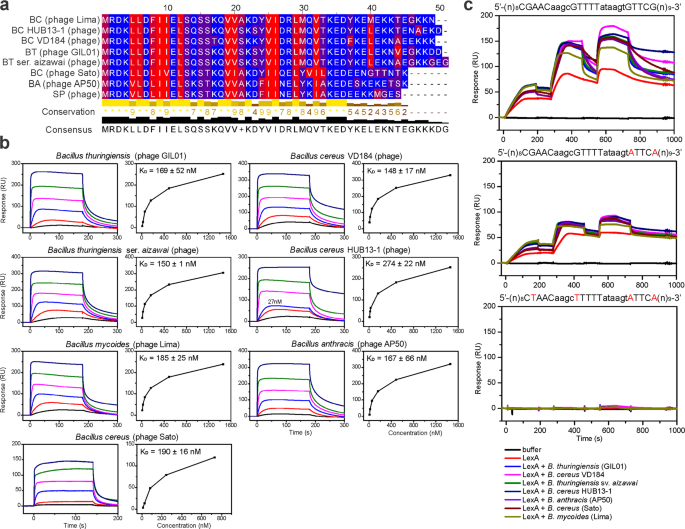
a Amino-acid sequences of tectiviral gp7 homologs aligned with Clustal Omega and visualized using Jalview. Amino acids are color coded according to the hydrophobicity scale, with red indicating hydrophobic residues, and blue indicating hydrophilic residues. b Surface plasmon resonance sensorgrams of the interaction of gp7 and gp7 homologs (respective hosts are indicated above the diagrams) with immobilized B. thuringiensis LexA. Analytes were injected at 3-fold serial dilutions (6–1458 nM) for 180 s at a flow rate of 30 μL min−1 over chip-immobilized LexA. Apparent equilibrium dissociation constants (KD) are expressed as means ± standard deviations of two titrations of each analyte. c Surface plasmon resonance sensorgrams of the interaction between B. thuringiensis LexA alone or pre-incubated with gp7 or gp7 homologs with the wild-type or mutant LexA operators from the GIL01 P1 promoter region16. Sequences of DNA fragments used are shown above the diagrams, with important nucleotides for LexA binding shown in capital letters, and changed nucleotides shown in red. Free LexA (5, 10, 20 nM) or LexA (5, 10, 20 nM) pre-incubated with gp7 and gp7-like proteins in 1:10 molar ratio (50, 100, 200 nM) were injected over the selected DNAs immobilized on the chip at 50 μL min−1 in a single cycle. The experiment was performed in duplicate and representative sensorgrams are shown.
Discussion
The data provided here show that a hierarchy has evolved in B. thuringiensis serovar israelensis between the two co-residential prophages carried on the phage-plasmid extrachromosomal elements pBtic235 and pGIL0130. Transcriptomic analysis of the interaction between B. thuringiensis and the two prophages revealed that the linear 15-kb prophage GIL01 represses more than 71% of the plasmid and phage module genes carried by pBtic235 in the DNA damage response. Remarkably, we showed that GIL01 deploys gp7 to control the phage-pBtic235 cycle. The transcriptomic data show that ectopic expression of gp7 at native levels down-regulates the pBtic235 genes in the presence of a prophage-inducing agent. Gp7 significantly repressed the production of phage pBtic235 when GBJ002, the pBtic235 lysogen, was exposed to DNA-damaging stress. In contrast to its role in GIL0114, we show here that gp7 is not crucial for establishing the phage-pBtic235 lysogenic cycle, and that the key determinants of pBtic235 required for quiescence appear to be located in the ~10-kb region, which is absent in a pBtic235 clear-plaque mutant. Thus, gp7 is a dual-function regulator that, depending on the environmental conditions, enables GIL01 to either enter the dormant state or be induced before the co-resident prophage pBtic235.
Gp7 acts as a master regulator as it down-regulated genes that constitute the core LexA-regulon in bacteria25,31, with roles in SOS regulation, DNA recombination and excision, and error-prone DNA repair. Unexpectedly, gp7 upregulated the genes for two cytolysins, a phospholipase C, and a collagenase in SOS-induced cells, which suggests that gp7 can influence the synthesis, and possibly the release, of B. thuringiensis virulence determinants32. However, the phage GIL01 appears to have evolved mechanisms beyond the gp7 regulator. We observed that in response to DNA damage, the prophage GIL01 not only inhibited the expression of the pBtic235 genes but also repressed the transcription of the host virulence factors, but did not affect the expression of the SOS genes. We hypothesize that certain GIL01 prophage determinants have co-evolved with gp7 to preserve the sensitivity of the cellular DNA-damage response, which is important for host survival, and to fine-tune certain host functions, such as virulence-factor expression, with the lytic life cycle processes of GIL01.
Although most bacteria carry multiple prophages33, the regulatory mechanisms that coordinate their induction at the transcription level remain largely unexplored. The food-borne pathogen Listeria monocytogenes has been shown to carry a co-regulated functional prophage and a cryptic phage element controlled by the same inducer5. A DNA-damage-inducible metalloprotease on the cryptic phage inactivates the cI-like repressors of both prophages, resulting in synchronized lytic induction. Interestingly, the induction of these prophage elements is attenuated by the prophage repressor AriS, which interferes with the host SOS response. However, the inhibitory effect of the SOS response was only observed when the AriS was ectopically overexpressed and not transcribed from the prophage34. This is consistent with our observation that in addition to gp7, other GIL01 factors also influence host gene expression. In addition, Salmonella enterica prophages Gifsy-1 and Gifsy-3 carry SOS-induced anti-repressors that also cause the simultaneous induction of prophages into the lytic cycle function by direct binding and the displacement of cognate and noncognate phage repressors bound at target DNA sites4. To the best of our knowledge, the present study is the first report of a small phage-borne protein that modulates transcriptional patterns in a cohabiting phage to extend its latency.
In E. coli, an as-yet-unidentified factor is required for LexA to bind noncanonical sequence motifs27, and in the multidrug-resistant pathogen Acinetobacter baumanii, the LexA-like repressor UmuDAb controls the DNA-damage-inducible genes through a direct interaction with the ~9-kDa co-repressor DdrR, although the mechanism is not yet fully understood35,36. Similar to the narrow distribution of gp7 homologs across the tectivirus genomes, DdrR is only found in the chromosomes of Acinetobacter spp.37. This suggests that the LexA accessory proteins have co-evolved with the LexA repressors of their hosts, and thus have different origins but function congruently. We anticipate that the present study will provide the impetus to explore the small gp7-like proteins of viral and bacterial origin that link the host DNA-damage responses and other processes to the prophage induction and the movement of co-resident mobile genetic elements between bacteria21,38,39. It is tempting to speculate that this 'know-how' hidden in the LexA accessory proteins might offer a way to manipulate pathogens.
Material and methods
Bacterial strains, plasmids, and culture conditions
All the strains and plasmids used in this study are listed and described in Supplementary Table 1. The B. thuringiensis strains were routinely cultured in a Lysogeny Broth (LB) medium at 30 °C and 180 rpm, unless otherwise indicated. The E. coli and S. aureus strains were grown in a LB at 37 °C and 180 rpm. For overnight cultures, a single bacterial colony grown on LB agar plates was inoculated into 5 mL of LB medium and incubated for 14 h to 16 h at 30 °C and 180 rpm. Exponential cultures were prepared by inoculating the overnight culture into a fresh medium in a ratio of 1:100. Kanamycin (Sigma) was added to the culture medium at 50 µg mL−1 for solid LB agar plates at 25 µg mL−1 for overnight cultures and 10 µg mL−1 for exponential cultures to select for plasmids pDG and pDG7. For the agar plates, the LB medium was solidified with 1.5% (w/v) agar and soft-agar overlays with 0.4% (w/v) agar. Soft agar overlays containing the strain B. thuringiensis HER1410 as a recipient host were prepared by mixing 200 µL of the culture in an early exponential phase with 5 mL of soft agar at 48 °C. The soft-agar mixture was then poured onto warm LB agar plates and left to solidify for 30 min at room temperature before spotting 10 µL serial dilutions of the phage stocks. Protein expression from the pDG7 plasmid was induced by adding 0.1 mM isopropyl-β-D-1 thiogalactopyranoside (IPTG; final concentration) to the cultures.
Sample preparation for RNA extraction and sequencing
Cultures for the transcriptome analysis were prepared by inoculating 150 mL of LB with an overnight culture of GBJ002, GBJ002(GIL01), GBJ002(pDG) or GBJ002(pDG7) in the ratio 1:100 and the addition of 0.1 mM IPTG to the GBJ002(pDG) and GBJ002(pDG7) strains. The cultures were grown aerobically at 30 °C for approximately 3 h, until an early exponential phase (OD595, 0.3-0.4). At this time, the cultures were divided into two and 100 ng mL−1 of MMC was added to one of the cultures. Culture samples (7 mL) of MMC-treated or untreated GBJ002 and GBJ002(GIL01) were taken 30 min after the addition of MMC, and of GBJ002(pDG) and GBJ002(pDG7) 1 h after the addition of MMC. Immediately after collection the culture samples were centrifuged at 15,000× g for 90 s and resuspended in 800 µL of DNA/RNA Shield reagent (Zymo Research, USA), which preserves the nucleic acids at room temperature. Samples were then transferred to lysis tubing (0.1, 0.5 mm; ZR BashingBead; Zymo Research) and mechanically homogenized by bead beating using a mixer mill (MM 301; Retsch) for 10 min at 30 Hz. The total RNA was isol...
Comments
Post a Comment