Protective effect of microbisporicin (NAI-107) against vancomycin resistant Enterococcus faecium infection in a Galleria ... - Nature.com
Abstract
Increasing antimicrobial resistance in Enterococcus faecium necessitates the search for novel treatment agents, such as bacteriocins. In this study, we conducted an in vivo assessment of five bacteriocins, namely Lacticin Z, Lacticin Q, Garvicin KS (ABC), Aureocin A53 and Microbisporicin (NAI-107), against vanB-resistant Enterococcus faecium using a Galleria mellonella model. Our in vitro experiments demonstrated the efficacy of all five bacteriocins against vanB-resistant E. faecium with only NAI-107 demonstrating in vivo efficacy. Notably, NAI-107 exhibited efficacy across a range of tested doses, with the highest efficacy observed at a concentration of 16 µg/mL. Mortality rates in the group treated with 16 µg/mL NAI-107 were lower than those observed in the linezolid-treated group. These findings strongly suggest that NAI-107 holds promise as a potential alternative therapeutic agent for treating infections caused by resistant E. faecium and warrants further investigation.
Similar content being viewed by others
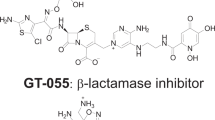
In vitro and in vivo activity of GT-1, a novel siderophore cephalosporin, and GT-055, a broad-spectrum β-lactamase inhibitor, against biothreat and ESKAPE pathogens
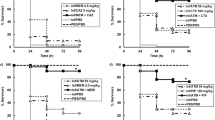
Dual β-lactam combination therapy for multi-drug resistant Pseudomonas aeruginosa infection: enhanced efficacy in vivo and comparison with monotherapies of penicillin-binding protein inhibition
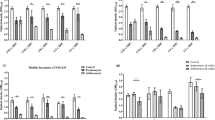
Comparison of solithromycin with erythromycin in Enterococcus faecalis and Enterococcus faecium from China: antibacterial activity, clonality, resistance mechanism, and inhibition of biofilm formation
Introduction
Enterococcus faecium and Enterococcus faecalis are Gram-positive facultative anaerobes that are commonly found in the gastrointestinal tracts of humans and animals1,2. Infections caused by vancomycin-resistant enterococci (VRE) emerged in Europe in the 1980's and are becoming an increasing problem worldwide1,3. The vast majority of VRE infections are caused by E. faecium. Surveillance data from the United States revealed that the prevalence of vancomycin resistance in E. faecalis and E. faecium was 7% and 80%, respectively4.
Typically, resistance to vancomycin in enterococci is due to the presence of the vanA or vanB genes5. They are situated on a transposon (Tn1546 for vanA, Tn1547, or Tn5382 for vanB)5. The vanA gene results in a high level of resistance to both vancomycin and teicoplanin, while vanB exclusively provides resistance to vancomycin6,7. Vancomycin-resistant E. faecium infections are particularly difficult to treat, given their resistance to beta-lactams1,8. Treatment options include linezolid, but combined linezolid-vancomycin resistance is possible9. Linezolid toxicity is a frequent problem, and the utility of this agent for certain types of infection, such as endocarditis, is uncertain8,9,10.
This paucity of treatment options for VRE infections provided the motivation for this study, where we aimed to assess if bacteriocins that show in vitro activity against VRE would exhibit this activity in vivo. Bacteriocins have gained considerable attention as potential alternatives to traditional antibiotics due to their narrow-spectrum activity and a lower likelihood of inducing resistance11,12. Most leaderless bacteriocins such as Lacticin Q (53 aa), Lacticin Z (53 aa), and Garvicin KS have proven to have inhibitory effects against a range of genera such as Listeria, Staphylococcus and Enterococcus13,14. The lantibiotic NAI-107 has been found to be active against a wide range of multi-drug resistant (MDR) Gram-positive bacterial pathogens, including methicillin resistant Staphylococcus aureus (MRSA), glycopeptide-intermediate resistant S. aureus (GISA) and vancomycin-resistant Enterococci (VRE)12,15,16. Additionally, NAI-107 was found to be effective against vanA E. faecium 569 and vanA E. faecalis A533 in vivo when administered intravenously to neutropenic mice17. In addition to bacteriocins, natural antimicrobial compounds or semi-synthetic derivatives of microbial natural products such as Nigella sativa oil extract, bacillusin A (23), Nicrophorusamide A, Urnucratin A have been shown to exhibit promising efficacy against VRE18,19,20.
G. mellonella has been shown to be an effective model host for investigating the efficacy of antimicrobial agents against various pathogens21,22. This model serves as a viable alternative to traditional mammalian in vivo systems. The immune response in Galleria mellonella encompasses both cellular mechanisms—such as phagocytosis—and humoral responses, which include melanization, hemolymph clotting, and the synthesis of antimicrobial peptides23,24,25. Crucially, studies have found that the efficacies of antimicrobial agents on infected G. mellonella larvae closely correlate with the known drug susceptibilities of the pathogens in vitro and in mammalian models26,27,28,29. In addition, the use of G. mellonella enables the application of the 3Rs principles (replacement, reduction and refinement) in animal experimentation29.
We therefore explored a panel of bacteriocins from PARAGEN collection (Syngulon) and Microbisporicin NAI-107 (Naicons, SRL) against vanB-resistant E. faecium in a G. mellonella model. The PARAGEN collection consists of 66 bacteriocins and four of these bacteriocins (Lacticin Z, Lacticin Q, Garvicin KS (ABC), Aureocin A53) showed in vitro antimicrobial activity against vanB-resistant E. faecium (Table 1)30.
Materials and methods
E. faecium strains and minimum inhibitory concentrations (MIC) of bacteriocins and antimicrobial drugs
VanB-type E. faecium (ID 553) was used for all the experiments. Sixty-six bacteriocins from Syngulon PARAGEN collection and NAI-107 from Naicons, SRL were screened using the resazurin microtiter assay (REMA)30,31. The MIC for the antimicrobials listed in Table 1 were determined according to the clinical and laboratory standard institute (CLSI) guidelines and the European Committee on Antimicrobial Susceptibility Testing (EUCAST) broth microdilution reference method for MIC determination in E. faecium32,33.
In vivo studies
- (a)
Determining the appropriate dose of E. faecium
G. mellonella larvae were treated with a range of doses of vanB-type E. faecium (ID 553) (1 × 106–1 × 109 CFU/mL) and observed for death alongside phosphate buffer saline (PBS) controls. The dose that resulted in approximately 80% mortality was 2.7 × 107 CFU in 30 µl PBS, which was further used in the G. mellonella infection model.
- (b)
Galleria mellonella infection model
Injection of G. mellonella was carried out as described in Dijokaite et al.21. The last larval stage of G. mellonella (Terramania, Arnhem, NL) were used for the experiments. Only non-discoloured, healthy larvae were selected. The larvae were injected in the last left proleg using 0.3 mL U-100 insulin syringes (BD Micro-Fine).
Each test group consisted of two groups of 10 larvae per concentration of each bacteriocin or linezolid (10 µg/mL). 30 µl of PBS containing 2.7 × 107 CFU E. faecium was used to infect the G. mellonella larva. The larvae were treated with various concentrations of bacteriocins. One positive and two negative control groups were used. The positive control group received only the E. faecium inoculum in PBS. Out of the two negative control groups, one group underwent no manipulation, while the other group was injected with PBS only. Each antimicrobial agent was tested in a single experiment using larvae from the same batch and tested at the same time under identical conditions. Each group were incubated in sterile Petri dishes at 37 °C with a 5% (v/v) CO2 atmosphere for the length of the experiments. The larvae were observed for 120 h for any indications of illness, necrosis, or paralysis, which enabled an evaluation of the bacteriocin's toxicity. Larvae were scored dead if they did not respond to touch stimuli by blunt sterile forceps and scored for 5 consecutive days (120 h).
An overview of the in vivo assay in Galleria mellonella model is shown in Fig. 1.
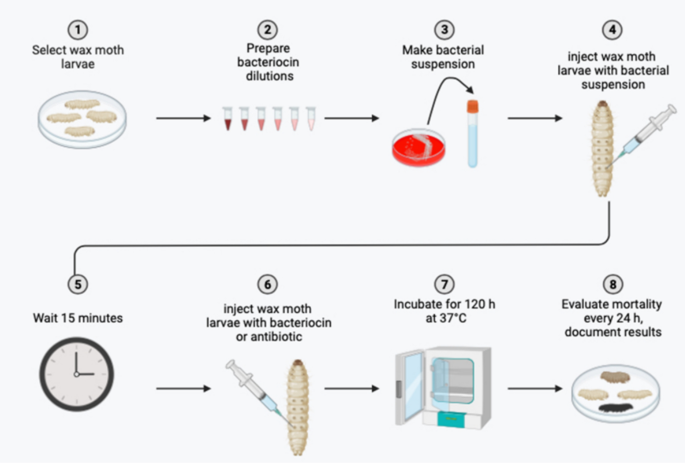
Overview of the in vivo assay in Galleria mellonella model. (Figure was generated using BioRender).
Statistical analysis
Data were analyzed using GraphPad Prism v9. Survival plots were created using Kaplan–Meier survival curves. Statistical analysis was carried out using the Mantel-Cox test to compare survival curves between the PBS controls and each treatment arm. A P value of < 0.05 was considered statistically significant.
Results
MIC determination
Five of the bacteriocins, Lacticin Q (LcnQ), LacticinZ (LcnZ), Aureocin A53 (AucA), Garvicin KS (ABC) and microbisporicin (NAI-107) had the highest antimicrobial activity against van-B type E. faecium and were selected for the in vivo experiments (Table 1, Supplementary Table S1). The MICs of the antimicrobials and bacteriocins are listed in Table 1.
In vivo assay for efficacy of bacteriocins in Galleria mellonella E. faecium infection model
The in vivo efficacy of A53, GarKS (ABC), lacticin Q, lacticin Z and NAI-107 were determined against vanB-type E. faecium in comparison to linezolid. Despite the in vitro activity of all five of these bacteriocins, four of the bacteriocins [A53, GarKS (ABC), lacticin Q and lacticin Z) did not demonstrate any detectable efficacy in vivo (Fig. 2).
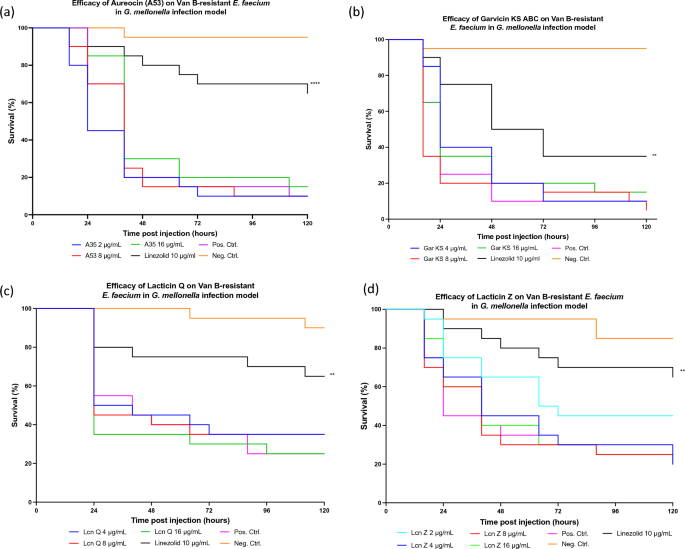
Efficacy of (a) Aureocin A53 (2, 8, and 16 µg/mL) and Linezolid (10 µg/mL) (b) Garvicin KS ABC (4, 8, and 16 µg/mL) (c) Lacticin Q (4, 8, and 16 µg/mL) and (d) Lacticin Z (2, 4, 8, and 16 µg/mL) treatment on G. mellonella larvae. A Negative control group was injected with 30 µL of PBS, and a positive control group was inoculated with 30 µL of PBS containing 2.7 × 107 CFUs of the E. faecium strain. Both test and control groups consisted of 20 larvae and were incubated for 120 h at 37 °C. Symbols represent mean survival of the G. mellonella. *P < 0.01, **P < 0.001, ***P < 0.0001, ****P < 0.00001.
NAI-107 was effective in vivo at all doses tested—2 µg/mL, 4 µg/mL, 8 µg/mL 16 µg/mL (P values 0.01 to < 0.0001; Fig. 3). Mortality was lowest in the 8 µg/mL and 16 µg/mL groups. The mortality in the 16 µg/mL was significantly lower than the linezolid treated group (P = 0.0221).
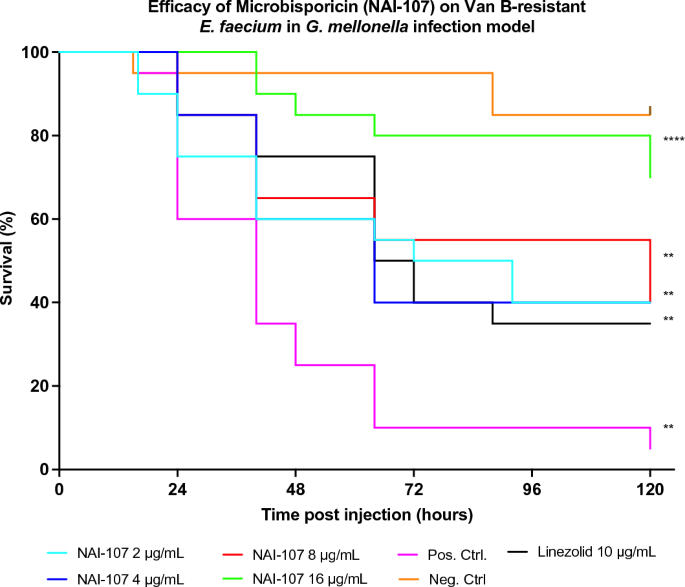
Effectiveness of Microbisporicin (2, 4, 8 and 16 µg/mL) and Linezolid (10 µg/mL) treatments on G. mellonella larvae. A negative control group was injected with 30 µL of PBS, and a positive control group was inoculated with 30 µL of PBS containing 2.7 × 107 CFUs of the E. faecium strain. Both test and control groups consisted of 20 larvae and were incubated for 120 h at 37 °C. Symbols represent mean survival of the G. mellonella. *P < 0.01, **P < 0.001, ***P < 0.0001, ****P < 0.00001.
Discussion
In our study, we observed that among the bacteriocins investigated, only NAI-107 demonstrated effectiveness in protecting Galleria mellonella from infection caused by vanB-type Enterococcus faecium. We do not have a definitive explanation for this difference, but several pharmacodynamic and pharmacokinetic factors may play a role. One of these is that all the bacteriocins, except NAI-107 may be metabolized by the G. mellonella29,34. Another possible explanation is that the mechanisms of action of these bacteriocins may differ. For example, the leaderless peptides such as Lacticin Q, typically form stable pores in bacterial membranes. In contrast, NAI-107 which are ribosomally synthesized peptides that undergo posttranslational modifications causes depolarization of the membrane resulting in bacterial cell death without the formation of stable pores35,36.
NAI-107 is a 23-amino acid lantibiotic (class I bacteriocin) produced by the actinomycete Microbispora sp. ATCC PTA-502412,35. Like other lantibiotics, it contains a methyllanthionine and three lanthionine bridges37. Unlike the other lantibiotics, it also contains two unusually modified amino acids: 5-chlorotryptophan and 3,4-dihydroxyproline37. It has novel mechanisms of action, which include the inhibition of lipid II mediated synthesis of peptidoglycan as well as disrupting inner cell membrane protein interactions leading to slow membrane depolarization (Supplementary Fig. S1)37. Importantly, NAI-107 exhibits no known cross resistance with other antimicrobials35. Previous studies have revealed a low risk for the emergence of resistance during therapy12. One study found that after 20 subpassages at increasing concentrations of NAI-107, NAI-107 MICs of N. gonorrhoeae, E. faecalis (vanA), E. faecium (vanA) and S. aureus (MRSA and GISA) increased only occasionally and to a maximum of 2 to fourfold the initial MIC12. Thus far the predominant resistance mechanism to lantibiotics detected, has been the production of an immunity protein by the producer strain12,35. Its novel mechanism of action is a likely explanation for the fact that no cross resistance between NAI-107 and other antimicrobials has been found35.
NAI-107 is not absorbed orally, but it does have good bioavailability after intramuscular, intravenous and subcutaneous administration1,38. In a mouse model, single subcutaneous dosing of 5, 20 and 80 mg/kg resulted in Cmax concentrations from 4 to 22 µg/ml, AUC values from 27 to 276 mg h/Liter, and elimination half-lives of 4.2–8.2 h39. This characteristic implies that NAI-107 would require parenteral administration in multiple doses per day for therapeutic use.
There are a number of study limitations. We only evaluated the effects of the bacteriocins on a single strain of vanB-type E. faecium and did not include vancomycin-sensitive E. faecium (VSE) strains as a control. The hypothesis we were testing in this study was that NAI-107 would exhibit activity in vivo against VRE. This hypothesis was motivated by the growing problem of VRE infections. We acknowledge the limitations of this hypothesis. Inclusion of VSE strains in our hypothesis would have allowed us to assess if NAI-107 shows promise for both VSE and VRE infections. This would be of clinical use as the vancomycin susceptibility of clinical isolates is not typically known when they are first identified. Further studies will be required to assess if NAI-107 is active against VSE strains. Our findings need to be replicated in a broader range of strains of E. faecium. As already noted, we did not investigate the reasons why four of the five bacteriocins were active in vitro but not in vivo. We acknowledge that there is considerable variation in the therapeutic efficacy of linezolid between the different experiments testing each antimicrobial compound. This may be explained by differences in the larvae used in the different experiments. Each compound was assessed in a single experiment conducted with the same batch of larvae but each subsequent experiments was conducted with a new batch of larvae. This makes comparisons between experiments inappropriate. Our results may be reproduced using different infection vertebrate and invertebrate models, such as those involving mice, rats, rabbits, dogs, zebrafish, Caenorhabditis elegans and Drosophila melanogaster (reviewed in40). However, Galleria mellonella has been successfully used to model various aspects of the colonization and infection of Enterococcus faecium40. Finally, we did not assess the toxicity of the bacteriocins as this has been done in previous studies27. NAI-107 exhibited no toxicity up to 64 µg/mL in a G. mellonella model27. Notably, NAI-107 has been found to be non-toxic and efficacious against methicillin-resistant Staphylococcus aureus in a rat model38.
If future studies confirm that NAI-107 is non-toxic and efficacious in human trials, it may emerge as a useful option for treating multi-resistant enterococcal infections, addressing a critical need in the field of antimicrobial therapy.
Data availability
All data generated or analysed during this study are included in this published article. Additional information can be addressed to the corresponding author upon reasonable request.
References
Uttley, A. et al. High-level vancomycin-resistant enterococci causing hospital infections. Epidemiol. Infect. 103(1), 173–181 (1989).
Fisher, K. & Phillips, C. The ecology, epidemiology and virulence of Enterococcus. Microbiology 155(6), 1749–1757 (2009).
Ramsey, A. M. & Zilberberg, M. D. Secular trends of hospitalization with vancomycin-resistant enterococcus infection in the United States, 2000–2006. Infect. Control Hosp. Epidemiol. 30(2), 184–186 (2009).
Hidron, A. I. et al. Antimicrobial-resistant pathogens associated with healthcare-associated infections: Annual summary of data reported to the National Healthcare Safety Network at the Centers for Disease Control and Prevention, 2006–2007. Infect. Control Hosp. Epidemiol. 29(11), 996–1011 (2008).
Ahmed, M. O. & Baptiste, K. E. Vancomycin-resistant enterococci: A review of antimicrobial resistance mechanisms and perspectives of human and animal health. Microb. Drug Resist. 24(5), 590–606 (2018).
Hashimoto, Y. et al. Amino acid substitutions in the VanS sensor of the VanA-type vancomycin-resistant Enterococcus strains result in high-level vancomycin resistance and low-level teicoplanin resistance. FEMS Microbiol. Lett. 185(2), 247–254 (2000).
Courvalin, P. Vancomycin resistance in gram-positive cocci. Clin. Infect. Dis. 42(Supplement_1), S25–S34 (2006).
Yadav, G. et al. Linezolid and vancomycin resistant enterococci: A therapeutic problem. J. Clin. Diagn. Res. JCDR 11(8), GC07 (2017).
Herrero, I. A., Issa, N. C. & Patel, R. Nosocomial spread of linezolid-resistant, vancomycin-resistant Enterococcus faecium. N. Engl. J. Med. 346(11), 867–869 (2002).
Vinh, D. C. & Rubinstein, E. Linezolid: A review of safety and tolerability. J. Infect. 59, S59–S74 (2009).
Marshall, S. H. & Arenas, G. Antimicrobial peptides: A natural alternative to chemical antibiotics and a potential for applied biotechnology. Electron. J. Biotechnol. 6(3), 271–284 (2003).
Brunati, C. et al. Expanding the potential of NAI-107 for treating serious ESKAPE pathogens: Synergistic combinations against Gram-negatives and bactericidal activity against non-dividing cells. J. Antimicrob. Chemother. 73(2), 414–424 (2018).
Ovchinnikov, K. V. et al. Novel group of leaderless multipeptide bacteriocins from gram-positive bacteria. Appl. Environ. Microbiol. 82(17), 5216–5224 (2016).
Chi, H. & Holo, H. Synergistic antimicrobial activity between the broad spectrum bacteriocin garvicin KS and nisin, farnesol and polymyxin B against gram-positive and gram-negative bacteria. Curr. Microbiol. 75, 272–277 (2018).
Castiglione, F. et al. Determining the structure and mode of action of microbisporicin, a potent lantibiotic active against multiresistant pathogens. Chem. Biol. 15(1), 22–31 (2008).
Maffioli, S. I. et al. Characterization of the congeners in the lantibiotic NAI-107 complex. J. Nat. Prod. 77(1), 79–84 (2014).
Almeida-Santos, A. C. et al. Enterococcus spp. as a producer and target of bacteriocins: a double-edged sword in the antimicrobial resistance crisis context. Antibiotics 10(10), 1215 (2021).
Yern-Hyerk, S. et al. Nicrophorusamides A and B, Antibacterial Chlorinated Cyclic Peptides from a Gut Bacterium of the Carrion Beetle Nicrophorus concolor (2017).
Ravu, R. R. et al. Bacillusin A, an antibacterial macrodiolide from Bacillus amyloliquefaciens AP183. J. Nat. Prod. 78(4), 924–928 (2015).
Ahmad, A. A. M. et al. Nigella sativa oil extract: A natural novel specific conjugal transfer inhibitor of vancomycin resistance from vanA/B-resistant Enterococcus faecium to Staphylococcus aureus. J. Appl. Microbiol. 133(2), 619–629 (2022).
Dijokaite, A. et al. Establishing an invertebrate Galleria mellonella greater wax moth larval model of Neisseria gonorrhoeae infection. Virulence 12(1), 1900–1920 (2021).
Gibreel, T. M. & Upton, M. Synthetic epidermicin NI01 can protect Galleria mellonella larvae from infection with Staphylococcus aureus. J. Antimicrob. Chemother. 68(10), 2269–2273 (2013).
Hoffmann, J. A. Innate immunity of insects. Curr. Opin. Immunol. 7(1), 4–10 (1995).
Jander, G., Rahme, L. G. & Ausubel, F. M. Positive correlation between virulence of Pseudomonas aeruginosa mutants in mice and insects. J. Bacteriol. 182(13), 3843–3845 (2000).
Evans, B. & Rozen, D. A Streptococcus pneumoniae infection model in larvae of the wax moth Galleria mellonella. Eur. J. Clin. Microbiol. Infect. Dis. 31, 2653–2660 (2012).
Six, A. et al. Galleria mellonella as an infection model for the multi-host pathogen Streptococcus agalactiae reflects hypervirulence of strains associated with human invasive disease. Virulence 10(1), 600–609 (2019).
Hofkens, N. et al. Microbisporicin (NAI-107) protects Galleria mellonella from infection with Neisseria gonorrhoeae. Microbiol. Spectr. 11(6), e02825-e2923 (2023).
Thomas, R. J. et al. Galleria mellonella as a model system to test the pharmacokinetics and efficacy of antibiotics against Burkholderia pseudomallei. Int. J. Antimicrob. agents 41(4), 330–336 (2013).
Pereira, M. F. et al. Galleria mellonella as an infection model: an in-depth look at why it works and practical considerations for successful application. Pathogens Dis. 78(8), ftaa056 (2020).
Gabant, P. & Borrero, J. PARAGEN 1.0: A standardized synthetic gene library for fast cell-free bacteriocin synthesis. Front. Bioeng. Biotechnol. 7, 213 (2019).
Martin, A. et al. Promising antimicrobial activity and synergy of bacteriocins against mycobacterium tuberculosis. Microb. Drug Resist. 29(5), 165–174 (2023).
Wayne, P. Clinical and Laboratory Standards Institute: Performance Standards for Antimicrobial Susceptibility Testing: 20th Informational Supplement. CLSI document M100-S20 (2010).
Weinstein, M. P. Comparative evaluation of penicillin, ampicillin, and imipenem MICs and susceptibility breakpoints for vancomycin-susceptible and vancomycin-resistant Enterococcus faecalis and Enterococcus faecium. J. Clin. Microbiol. 39(7), 2729–2731 (2001).
Tsai, C.J.-Y., Loh, J. M. S. & Proft, T. Galleria mellonella infection models for the study of bacterial diseases and for antimicrobial drug testing. Virulence 7(3), 214–229 (2016).
Münch, D. et al. The lantibiotic NAI-107 binds to bactoprenol-bound cell wall precursors and impairs membrane functions. J. Biol. Chem. 289(17), 12063–12076 (2014).
Yoneyama, F. et al. Peptide-lipid huge toroidal pore, a new antimicrobial mechanism mediated by a lactococcal bacteriocin, lacticin Q. Antimicrob. Agents Chemother. 53(8), 3211–3217 (2009).
Cruz, J. C. S. et al. Brominated variant of the lantibiotic NAI-107 with enhanced antibacterial potency. J. Nat. Prod. 78(11), 2642–2647 (2015).