Bacterial pathogens deliver water- and solute-permeable channels to plant cells - Nature.com
Abstract
Many animal- and plant-pathogenic bacteria use a type III secretion system to deliver effector proteins into host cells1,2. Elucidation of how these effector proteins function in host cells is critical for understanding infectious diseases in animals and plants3,4,5. The widely conserved AvrE-family effectors, including DspE in Erwinia amylovora and AvrE in Pseudomonas syringae, have a central role in the pathogenesis of diverse phytopathogenic bacteria6. These conserved effectors are involved in the induction of 'water soaking' and host cell death that are conducive to bacterial multiplication in infected tissues. However, the exact biochemical functions of AvrE-family effectors have been recalcitrant to mechanistic understanding for three decades. Here we show that AvrE-family effectors fold into a β-barrel structure that resembles bacterial porins. Expression of AvrE and DspE in Xenopus oocytes results in inward and outward currents, permeability to water and osmolarity-dependent oocyte swelling and bursting. Liposome reconstitution confirmed that the DspE channel alone is sufficient to allow the passage of small molecules such as fluorescein dye. Targeted screening of chemical blockers based on the predicted pore size (15–20 Å) of the DspE channel identified polyamidoamine dendrimers as inhibitors of the DspE/AvrE channels. Notably, polyamidoamines broadly inhibit AvrE and DspE virulence activities in Xenopus oocytes and during E. amylovora and P. syringae infections. Thus, we have unravelled the biochemical function of a centrally important family of bacterial effectors with broad conceptual and practical implications in the study of bacterial pathogenesis.
Similar content being viewed by others
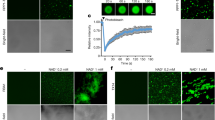
Substrate-induced condensation activates plant TIR domain proteins
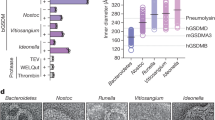
Structure and assembly of a bacterial gasdermin pore
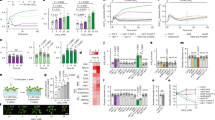
Mechanisms of calcium homeostasis orchestrate plant growth and immunity
Main
All AvrE/DspE-family effectors examined, including AvrE from P. syringae, WtsE from Pantoea stewartii, DspA/E (DspE hereinafter) from E. amylovora and DspE from Pectobacterium carotovorum, are major virulence factors responsible for bacterial multiplication and induction of major disease symptoms including water soaking and host cell death during infection7,8,9,10,11,12,13,14,15,16,17,18,19. AvrE-family effectors have been challenging to study owing to their extremely large size (approximately 200 kDa), high toxicity to plant and yeast cells and the fact that they share few sequence similarities with proteins of known function20,21. Several AvrE-family effectors were reported to interact with plant proteins, including plant protein phosphatase PP2A subunits, type one protein phosphatases and receptor-like kinases22,23,24,25. In addition, a yeast cdc55 mutation affecting Cdc55-PP2A protein phosphatase activity was found to suppress DspE-induced yeast growth arrest21. Although these interactions associate AvrE-family effectors with various host cellular processes, the fundamental question regarding the actual biochemical function of AvrE-family effectors has remained elusive.
In this study, we carried out AlphaFold2 analysis of the three-dimensional models of AvrE-family proteins. Unexpectedly, AlphaFold2 predicts that this family of proteins fold into a porin-like β-barrel structure. This prediction prompted us to conduct a series of cryogenic electron microscopy (cryo-EM) imaging, Xenopus oocyte, liposome and in planta experiments. Our results show that AvrE-family effectors are water- and solute-permeable channels that can be blocked by polyamidoamine (PAMAM) dendrimers. The discovery of the water- and solute-permeable channel function of AvrE/DspE-family effectors solves a decades-long puzzle regarding one of the most important families of phytobacterial type III effectors and marks a major advance in understanding bacterial pathogenesis.
AlphaFold2 analysis and cryo-EM imaging
To gain functional insights into the AvrE family of bacterial effectors, we constructed their three-dimensional models predicted by AlphaFold226 using the fast homology search of MMseqs2 (ColabFold)27. The predicted AlphaFold2 models of DspE from E. amylovora, DspE from P. carotovorum, AvrE from P. syringae pv. tomato (Pst) DC3000 and WtsE from P. stewartii (Fig. 1 and Extended Data Figs. 1 and 2) all reveal an overall similar architecture resembling a mushroom, with a prominent central β-barrel forming the stem, which is surrounded by a globular amino-terminal domain (E. amylovora DspE: K298–H672), a WD40 repeat domain (H673–P912) and two perpendicularly arranged helix bundles (E998–T1222 and A1567–H1647) on the top. The predicted domain arrangement is supported by our cryo-EM imaging of E. amylovora DspE, for which the two-dimensional class averages clearly reveal an overall similar top view to that of the AlphaFold model, with circularly arranged globular domains surrounding a central pore (Fig. 1a,b).
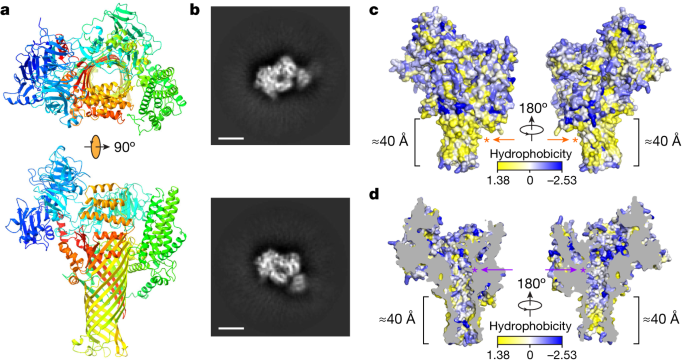
a, Three-dimensional model of E. amylovora DspE generated by AlphaFold2 using MMseqs2 (ColabFold). DspE (residues 298–1838) is shown in the cartoon model in a rainbow colour gradient, with the N terminus in blue and the C terminus in red. b, Cryo-EM two-dimensional class averages of DspE, revealing a circular arrangement of domains around a pore. Scale bars, 5 nm. The result is representative of three experimental replicates. c, Surface representation of DspE. d, Sliced view of DspE. In c,d, residues are coloured on the basis of their hydrophobicity scale. The length of the proposed membrane-spanning β-barrel stem is labelled. Orange and purple asterisks mark the approximate locations of the hydrophobic cluster of L1776, L1777 and L1778 and the basic cluster of K1399 and K1401, respectively.
Further examination of the predicted E. amylovora DspE three-dimensional model reveals that surface β-barrel residues facing outside are enriched with hydrophobic amino acids (Fig. 1c), whereas inward-facing pore residues are predominantly hydrophilic (Fig. 1d). The length of the lower portion of the β-barrel stem covered by hydrophobic residues is estimated to be about 40 Å, roughly the thickness of a cellular membrane. As AvrE has previously been reported to be membrane anchored20, the β-barrel stem of AvrE-family effectors probably inserts into the membrane and functions as a channel, similar to that of bacterial porins28. Such a mode of insertion is distinct from that of pore-forming bacterial toxins, such as staphylococcal α-haemolysin and Clostridium perfringens β-toxin, for which the β-barrel is assembled through oligomerization of two long β-strands29,30.
DspE and AvrE currents in Xenopus oocytes
The prediction that AvrE-family effectors are channel-forming proteins prompted us to conduct experiments to test the hypothesis that AvrE and DspE may allow ion conductance when expressed in Xenopus oocytes. As shown in the current–voltage relationship (Fig. 2b,c), inward currents and outward currents at negative and positive test potentials, respectively, were detected from oocytes injected with dspE or avrE complementary RNA (cRNA). The reversal potentials for DspE and AvrE channels are approximately −25 mV. The currents were not affected by niflumic acid, which blocks an endogenous Ca2+-activated Cl− channels31, or fipronil, which inhibits GABA-gated Cl− channels and glutamate-gated Cl− channels32 (Extended Data Fig. 3a,b). Surface biotinylation experiment with oocytes expressing DspE confirmed that this protein is anchored across the oocyte membrane (Extended Data Fig. 4a).
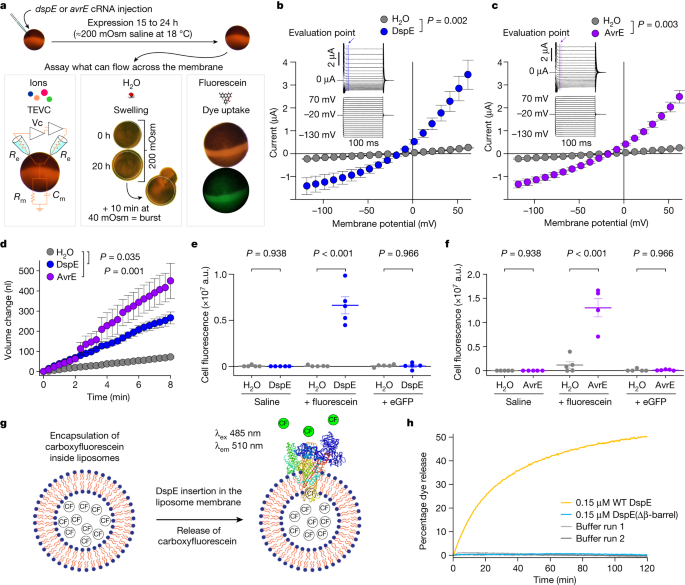
a, Schematic of the three oocyte assays, showing details for the two-electrode voltage clamp (TEVC) to test ion conductance (bottom left) (Vc, voltage command; Re, electrode resistance; Rm, membrane resistance; and Cm, membrane capacitance), the baseline and induced swelling and burst assay to test water conductance (bottom middle) and dye uptake to test conductance to molecules larger than single ions (bottom right). b,c, DspE and AvrE induce ion currents in the two-electrode voltage clamp assay. Mean ± s.e.m. (n = 5 oocytes) current values at different test pulses from oocytes expressing DspE (0.01 ng cRNA per oocyte) or AvrE (0.1 ng cRNA per oocyte) were recorded. d, DspE (2 ng) and AvrE (20 ng) induced fast oocyte swelling and burst at 24 h after cRNA injection when placed in a low-osmolarity (40 mOsm) solution. The data are presented as mean ± s.e.m. (n = 5 oocytes) of increased oocyte volume in relation to its initial volume. e,f, Fluorescein or eGFP entry assays. Oocytes injected with 2 ng dspE (e) or 20 ng avrE (f) cRNA or injected with water were incubated for 20 h in bath saline with or without fluorescein or eGFP. Values of fluorescence intensity were subtracted from the background and are presented as mean ± s.e.m. (n = 5 oocytes) corrected 'total cell fluorescence'. a.u., arbitrary units. g, Schematic of DspE-dependent release of carboxyfluorescein (CF) encapsulated within a liposome. h, Fluorescence increased over time for carboxyfluorescein-loaded liposome after addition of wild-type (WT) DspE (yellow), DspE(Δβ-barrel) (blue) or buffer (grey). The result is representative of three experimental replicates. P values were calculated using two-way analysis of variance (ANOVA; b,c,e,f) or two-way repeated measures ANOVA (d).
Source data
We further characterized whole-cell currents from DspE-expressing oocytes by conducting ion permeability experiments. Replacing extracellular sodium in ND96 recording solution (Methods) with potassium or other cations caused only minor variations in the magnitude of DspE currents (Extended Data Fig. 3c). Similarly, only minor variations in the magnitude of DspE currents were observed when extracellular Cl− was replaced with various anions, except for 4-morpholineethanesulfonic acid, sodium salt (Na-MES; Extended Data Fig. 3d,e). When 50% or 100% of the NaCl was replaced by Na-MES, progressively smaller outward currents were observed, and the reversal potential was shifted to a less negative value (Extended Data Fig. 3e). However, the negative reversal potential was not affected by replacement of other ions. These results suggest that Cl− may have a major role in carrying the outward current and that the DspE channel seems to have some selectivity towards anions including Cl−. Future research is needed to comprehensively survey possible ion selectivity of these channels.
DspE and AvrE induce cell swelling
During voltage-clamp current recording experiments, we noticed that many oocytes injected with dspE or avrE cRNA showed baseline swelling (Extended Data Fig. 5a), reminiscent of oocytes expressing plant aquaporins33. This raised the possibility that AvrE and DspE proteins may function like aquaporin channels to allow water to pass through cell membranes along an osmotic gradient, assuming that the osmolarity of the oocyte bathing medium (about 200 mOsm) may be lower than that of the oocyte cytoplasm. To more directly test this possibility, we adopted an oocyte swelling assay used for aquaporins and transferred oocytes from 200 mOsm to 40 mOsm bathing medium to create a larger osmotic difference. Notably, both DspE- and AvrE-expressing oocytes markedly swelled (Fig. 2d) and eventually burst (Supplementary Videos 1 and 2). We conducted further experiments to determine whether plant cells expressing AvrE would swell using our previously produced transgenic DEX::avrE plants20. As shown in Extended Data Fig. 5b,c, Arabidopsis leaf protoplasts expressing AvrE swelled to a greater extent compared to control Arabidopsis leaf protoplasts that have endogenous aquaporins. This provides further evidence that the AvrE channel has an ability to increase water permeability in planta7,8,9,10,11,12,13,14,15,16,17,18,19.
DspE channel size selectivity
The predicted AvrE/DspE channels have a diameter of 15–20 Å, much larger than the size of a water molecule or a simple ion. We reasoned that, in addition to ions and water, the AvrE and DspE channels may allow larger molecules to pass through. We conducted fluorescent dye permeability assays to test whether molecules smaller than the predicted pore size could pass through the membrane, whereas molecules larger than the predicted pore size could not. Two fluorescent molecules were tested: fluorescein (molecular mass of 332 Da with an estimated maximum molecular diameter of 7 Å) and enhanced green fluorescent protein (eGFP; molecular mass of 27 kDa with an estimated minimum diameter of 30 Å). As shown in Fig. 2e,f, fluorescein entered oocytes expressing DspE or AvrE, whereas eGFP could not, consistent with the predicted AvrE/DspE channel diameter. Notably, liposome-based in vitro reconstitution of DspE was sufficient to cause time-dependent release of carboxyfluorescein (molecular mass of 376 Da) encapsulated within soybean liposomes, whereas neither the buffer control nor the DspE(Δβ-barrel) mutant, in which most β-barrel-forming sequences are deleted, exhibited substantial activity (Fig. 2g,h), suggesting that no other protein is needed for baseline passage of small molecules through the DspE channel. As in the oocyte experiment, although DspE readily induced carboxyfluorescein dye release from liposomes (Fig. 2h), no time-dependent release of large molecules, such as fluorescein isothiocyanate–polysucrose 40 (molecular mass of 30–50 kDa with an estimated diameter of 80 Å), was observed (Extended Data Fig. 5d).
Mutational analysis of the DspE channel
We made several mutant derivatives of DspE to evaluate their functional consequences. As a negative control, DspE(Δβ-barrel) failed to induce water-soaking symptoms in Nicotiana benthamiana leaves, conduct ion currents, cause oocyte swelling or allow fluorescein release in the liposome assay (Fig. 2h and Extended Data Fig. 6). Similarly, triple substitution of three conserved hydrophobic, outward-facing residues (L1776, L1777 and L1778) of the predicted transmembrane region of DspE (location indicated by the orange asterisk in Fig. 1c) also abolished the DspE activities (Extended Data Fig. 6). Surface biotinylation of oocytes expressing DspE(Δβ-barrel) and DspE(L1776E/L1777E/L1778E) showed that they are no longer accessible by surface biotinylation and therefore probably cannot anchor across the membrane (Extended Data Fig. 4a). Finally, charge-reversal double substitution at K1399 and K1401, two inward-facing residues of the β-barrel (location indicated by the purple asterisk in Fig. 1d; not conserved among AvrE-family members), partially abolished the DspE activities (Extended Data Fig. 6). As a newly discovered family of channels, future research is needed to comprehensively define inward-facing residues that are critical for the function of DspE and other AvrE-family members.
Inhibitors of DspE and AvrE channels
Next, we attempted to identify compounds of molecular diameters that could fit the predicted pores of AvrE/DspE channels and might therefore block AvrE/DspE activities. We focused on a class of synthetic PAMAM dendrimers, which have programmable molecular diameters34. For example, PAMAM G0 has a diameter of 15 Å, whereas PAMAM G1 has a diameter of 22 Å (https://www.dendritech.com/pamam.html). Notably, the currents passing through the DspE and AvrE channels were reduced by G1 in a dose-dependent manner, reaching 71% inhibition on the DspE channel and 93% inhibition on the AvrE channel, respectively, at 10 mM G1 at 50 mV test pulse (Fig. 3a,b), while not affecting DspE expression (Extended Data Fig. 4c). Similar inhibition by G0 was observed on oocytes expressing DspE, reaching 68% inhibition at 10 mM G0 at 50 mV test pulse (Extended Data Fig. 7a).
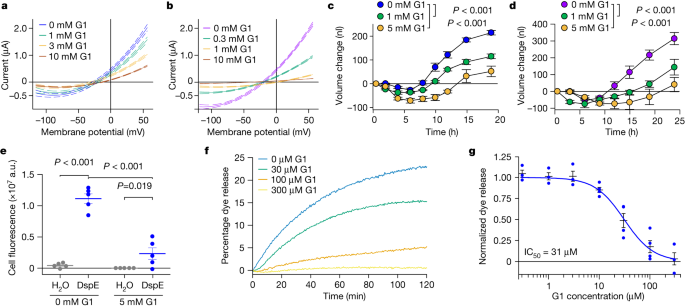
The assays were carried out according to Fig. 2a,g, except with inhibitors added to the bath saline. a,b, DspE-dependent (a) and AvrE-dependent (b) currents were inhibited by G1. Solid lines represent fit values for current (µA) across the entire voltage range, with dashed lines representing the lower and upper 95% confidence interval of a quadratic polynomial regression for each treatment after subtracting control values. See Extended Data Fig. 4c for DspE expression in G1-containing ND96. c,d, Baseline swelling of oocytes injected with 1 ng dspE (c) or 20 ng avrE (d) cRNA was reduced in the presence of G1. Data show mean ± s.e.m. (n = 5 oocytes) increase in volume from the start point (at the time of injection) after subtracting control values. e, Inhibition of fluorescein uptake. G1 reduced fluorescein entry into oocytes expressing DspE as evaluated 6 h after injection with 1 ng dspE per oocyte. Data are presented as in Fig. 2e. f, DspE-mediated dye release from liposomes in the presence of increasing concentrations of G1. The result is representative of three experimental replicates. g, Dose-dependent inhibition of liposome dye release. IC50, half-maximal inhibitor concentration. Data show mean ± s.e.m. (n = 3 batches of liposome preparations for 0.3, 1, 3 and 300 µM G1; n = 4 batches of liposome preparations for 10, 30 and 100 µM G1). Experiments were independently carried out two times for a,b,e, four times for c,d, and seven times for f,g. P values were calculated using Two-way ANOVA (e) or two-way repeated measures ANOVA (c,d).
Source data
When G1 was added to the ND96 incubation buffer, the baseline swelling over time was also inhibited in a dose-dependent manner, reaching a maximum of 76% inhibition for 5 mM G1 at 19 h after injection (Fig. 3c). Again, a similar effect was observed when this inhibitor was tested on AvrE-expressing oocytes, with 89% inhibition for 5 mM G1 (Fig. 3d).
We also tested the effect of G1 on fluorescein uptake by oocytes expressing DspE, and found that it inhibited fluorescent dye uptake, reaching 79% inhibition by the end of the assay (Fig. 3e). Finally, we tested G1 on purified DspE protein reconstituted in liposomes using the DspE-dependent liposome dye release assay. We found that G1 dose-dependently inhibited the release of fluorescein from the soybean liposomes after the addition of DspE (Fig. 3f). Fitting of the dye release yielded an IC50 value of 31 µM for G1 (Fig. 3g). Together, these findings show that we have identified PAMAM G1 as an inhibitor of AvrE/DspE-family channels.
PAMAM G1 inhibits bacterial infection
The ability of PAMAM G1 to inhibit DspE and AvrE activities in oocytes and liposomes in vitro raised the possibility that we had identified a lead compound that could interfere with the AvrE and DspE virulence function in planta during bacterial infection. We first tested this possibility against the AvrE function during P. syringae infection. In many P. syringae strains, AvrE is functionally redundant to another effector, HopM1 (refs. 11,13,19). Mutation of either avrE or hopM1 alone does not strongly affect Pst DC3000 virulence, but the avrE,hopM1 double mutant is severely impaired in virulence11,19. Notably, whereas avrE-family effector genes are conserved widely35, hopM1-family genes and/or their secretion chaperone genes are subjected to natural genetic mutations as in the case of the major pandemic bacterial pathogen P. syringae pv. actinidiae36. We found that G1 effectively inhibited Pst DC3000 infection of Arabidopsis in an AvrE-dependent manner (that is, inhibition occurs in the hopM1-deletion mutant, which simulates natural mutations in the hopM1 gene; Fig. 4a,b). Furthermore, inhibition of AvrE function by PAMAM G1 was not associated with induction of the PR1 protein, a marker for activation of salicylic acid-dependent immune responses in plants (Fig. 4c), or with negative effects on plant appearance (Extended Data Fig. 7b) or seed production (Extended Data Fig. 7c). Next we tested PAMAM G1 against E. amylovora infection. In E. amylovora, DspE plays an essential role in causing the devastating fire blight diseases, as the dspA/E mutant is largely nonpathogenic7,8. We found that G1 completely inhibited E. amylovora infection of highly susceptible pear fruits, phenocopying the dspE mutant of E. amylovora, an observation consistent with DspE being an indispensable virulence effector (Fig. 4d).
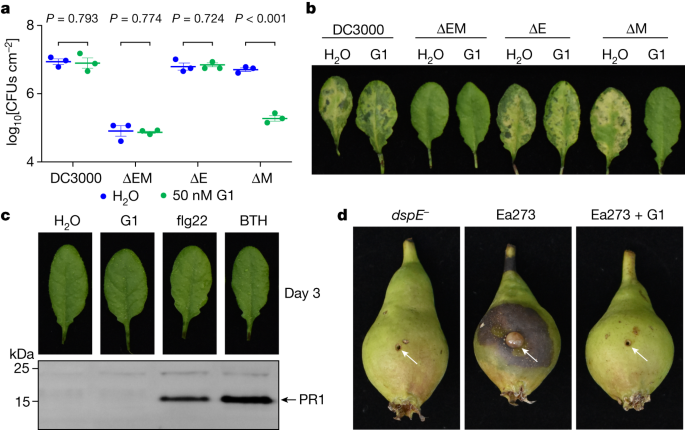
a,b, PAMAM G1 inhibits Pst DC3000 multiplication in an AvrE-dependent manner. A total of 1 × 106 colony-forming units (CFUs) per millilitre of Pst DC3000, the avrE,hopM1 double deletion mutant (ΔEM), the avrE single deletion mutant (ΔE) or the hopM1 single deletion mutant (ΔM) were syringe-inoculated into leaves of Arabidopsis WT Col-0 plants, with or without 50 nM PAMAM G1. Populations of bacteria (mean ± s.e.m.; n = 3 leaf samples) (a) in leaves were determined at day 3 after infiltration. Disease symptom pictures (b) were taken at day 4 after infiltration. c, PAMAM G1 does not induce PR1 protein expression in Arabidopsis. Arabidopsis Col-0 leaves were syringe-infiltrated with 10 µM PAMAM G1. Plants were kept under high humidity (>95%) for 3 days at 23 °C. PR1 protein in leaves was detected by an anti-PR1 polyclonal antibody. As positive controls, 100 µM benzothiadiazole (BTH), a synthetic chemical analogue of salicylic acid, and 1 µM flg22, a synthetic peptide derived from the conserved N-terminal 22 amino acids of bacterial flagellin, induce PR1 expression. The uncropped gel image is shown in Supplementary Fig. 1g. d, PAMAM G1 inhibits fire blight disease by E. amylovora Ea273. Immature pear fruits were spot-inoculated (indicated by arrows) with 10 µl of 1 × 103 CFUs ml−1 of Ea273 or the dspE mutant (dspE−), with or without 10 µM PAMAM G1. Inoculated pears were placed on a wet paper towel in a sterile box and incubated at 28 °C for 10 days. Diseased pears show areas with a dark, necrotic appearance. Experiments were carried out three times with similar results. P values were calculated using two-way ANOVA (a).
Source data
It is well known that type III secretion systems and their effectors are expressed and needed for bacterial growth only in host tissues, but not in vitro1,2. In accordance with this, G1 did not inhibit E. amylovora or Pst DC3000 growth in vitro (Extended Data Fig. 7d), providing further evidence that PAMAM G1 is truly a AvrE and DspE-specific virulence inhibitor, not just a nonspecific bactericidal antibiotic.
Discussion
Since the initial report of AvrE in P. syringae almost three decades ago37, the central importance of AvrE-family effectors in diverse pathogenic bacterial species has attracted the attention of researchers. Before this study, however, researchers largely pursued a working hypothesis that, like most other type III effectors, AvrE/DspE-family effectors would target host proteins, RNA or DNA to exert their virulence functions. Our finding that AvrE-family effectors could directly function as water- and solute-permeable channels in this study is therefore striking and unexpected. We propose a new integrated model for the function of AvrE-family effectors (Extended Data Fig. 8). The carboxy-terminal halves of AvrE-family effectors act primarily as a new class of water- and solute-permeable channels dedicated to creating osmotic/water potential perturbation and a water- and nutrient-rich apoplast in which bacteria multiply within the infected plant tissues. Future research is needed to determine how AvrE-family channel activity, discovered in this study, mediates water, solute and nutrient flows and apoplast osmolarity in planta to generate macroscopic water soaking, host cell death and defence suppression in the infected tissue, as shown previously6,13,19,25,38,39. We found that infiltrating the Arabidopsis leaf apoplast with water (to simulate water soaking) was sufficient to suppress flg22-induced callose deposition (Extended Data Fig. 9a,b), suggesting that water soaking and suppression of certain immune responses (for example, defence-associated callose deposition) are linked processes.
As large proteins with potentially many protein-interacting interfaces, AvrE-family effectors can additionally engage host proteins, including plant protein phosphatase PP2A subunits, type one protein phosphatases and receptor-like kinases22,23,24,25, to affect aspects of AvrE/DspE functions. It is striking that the AvrE channel inhibitor PAMAM G1 can essentially phenocopy the avrE or dspE genetic mutations in abrogating all major virulence phenotypes associated with AvrE and DspE, including water soaking, tissue necrosis and bacterial multiplication in infected tissues (Fig. 4). Therefore, future research should examine the possibility that some of the identified AvrE, DspE- and WtsE-interacting host proteins may act through modulating AvrE-family channel properties and/or optimizing downstream pathogenic outcomes of AvrE-family channel activities.
In summary, this study has unravelled the long-sought-after function of AvrE-family effectors. In addition, a chemical inhibitor of AvrE-family channels has been identified that appears to be broadly effective in reducing AvrE- and DspE-mediated bacterial infections. As such, the discovery of the water- and solute-permeable channel function of AvrE-family effectors has broad implications in the study of bacterial pathogenesis and bacterial disease control in plants.
Methods
Cloning, expression and purification of E. amylovora DspE and DspE(Δβ-barrel) in Escherichia coli
The dspE gene was PCR-amplified from the genomic DNA of E. amylovora strain Ea273 using the KOD hot start polymerase (Millipore Sigma) and the following primer set: DspE_Forward_primer: 5′-ATGGAATTAAAATCACTGGGAACTGAACACAAG -3′; DspE_Reverse_primer: 5′-GCTCTTCATTTCCAGCCCTTCCTTC-3′. The PCR product was cloned into a modified pET28a vector (Millipore Sigma) as a C-terminal fusion to maltose-binding protein (MBP) containing a His8 tag, a preScission protease cleavage site (PPX) and a Flag tag in the form of MBP–His8–PPX–Flag–DspE (hereinafter as MBP–DspE). Plasmid-transformed BL21(DE3) E. coli cells were grown in Luria–Bertani medium at 37 °C until the optical density at 600 nm (OD600 nm) reached 0.4–0.6, and were then induced with 0.1 mM IPTG and grown at 18 °C overnight. Collected cell pellets were resuspended in a lysis buffer containing 20 mM HEPES (pH 7.5), 300 mM NaCl and 2.5% glycerol, supplemented with cOmplete EDTA-free protease inhibitor tablet (Roche) and DNase I, and lysed by a French press. Following centrifugation at 20,000 r.p.m. for 30 min at 4 °C to remove cell debris, the fusion protein was purified using TALON Cobalt resin (Takara Bio). Following extensive washing in a buffer containing 20 mM HEPES (pH 7.5), 300 mM KCl, 2.5% glycerol, 1 mM ATP, 5 mM MgCl2 and 10 mM imidazole, the fusion protein was eluted in a buffer containing 20 mM HEPES (pH 7.5), 300 mM NaCl, 2.5% glycerol and 250 mM imidazole, and was further purified on a Superose 6 Increase 10/300 GL column (Cytiva Life Science) pre-equilibrated with a buffer containing 20 mM HEPES (pH 7.5), 150 mM NaCl and 1 mM dithiothreitol (DTT) at 4 °C. The protein fractions at the peak were aliquoted and flash-frozen at −80 °C for storage.
The construct of DspE(Δβ-barrel) (Δ1278–1566 + Δ1649–1813) was generated from the WT MBP–DspE construct described above through in-fusion cloning. The mutant protein was purified by following identical procedures as for the WT protein.
Representative size-exclusion chromatography profiles and SDS–polyacrylamide gel electrophoresis (PAGE) gels of the purified MBP–DspE and MBP–DspE(Δβ-barrel) proteins are shown in Extended Data Fig. 4g,h.
Constructs of MBP–DspE and MBP–DspE(Δβ-barrel) were made by the in-fusion cloning methods using the NEBuilder HiFi DNA assembly kit (New England Biolabs) with the following primer sets—WT DspE, dspE_forward_primer: 5′-GATGGAATTAAAATCACTGGGAACTGAACACAAG-3′; dspE_reverse_primer: 5′-GAAGGAAGGGCTGGAAATGAAGAGCTAATTGATTAA-3′; Vector_forward_primer: 5′-GAGCTAATTGATTAATACCTAGGCTGCTAAACAAAG-3′; Vector_reverse_primer: 5′-TTCTGTTCCAGGGGCCCGCGATGGAATTAAAATC-3′; DspE(Δβ-barrel) (Δ1278–1566 + Δ1649–1813), dspE forward_primer: 5′-CCTGGACAGTGCGGAGCCGGTGACCAGCAA-3′; dspE reverse_primer: 5′-AGGCTGCGGACAGCCACAGCGGAATAGCT-3′; Vector_forward_primer: 5′-CACAGCGGAATAGCTCAGGCTAATCCGCAG-3′; Vector_reverse_primer: 5′-GAATACGCTGTTGTCCCTGGACAGTGCGGA-3′.
Cryo-EM sample preparation and data collection and processing
Cryo-EM grids were prepared using a Leica EM GP2 automatic plunge freezer in a humidity-controlled chamber operated at 10 °C and 85–90% relative humidity. Homemade gold Quantifoil R1.2/1.3 300-mesh grids were glow-discharged using the PELCO easiGlow glow discharge cleaning system (TED PELLA) before sample application. During sample freezing, a 3-μl sample of DspE (about 1.1 mg ml−1) was applied to freshly glow-discharged grids and incubated on grids for 60 s before blotting with Whatman #1 filter paper for 2.8 s. The grids were then immediately plunge-frozen in liquid ethane and stored in liquid nitrogen before data acquisition.
A total of 7,810 micrograph stacks were recorded on an FEI Titan Krios electron microscope (Thermo Fisher) operated at 300 kV equipped with a K3 direct electron detector (Gatan) operated in the counting mode. Micrograph stacks were collected at a nominal magnification of ×81,000 using a pixel size of 1.08 Å per pixel with a defocus range from −2.4 μm to −0.8 μm using the Latitude S (Version 3.51.3719.0, Gatan) automated image acquisition package. Each stack was exposed for 2.8 s with an exposure time of 0.047 s per frame, resulting in 60 frames per stack. The total dose was approximately 56.3 e− Å−2 for each stack.
Motion correction and contrast transfer function (CTF) estimation were carried out with the patch motion correction model and patch CTF estimation module in cryoSPARC40. A total of 7,141 micrographs were selected from a total of 7,810 images on the basis of the CTF fitting resolution using a cutoff value of 4.0 Å. A total of about 2.4 million particles were picked using pre-trained TOPAZ41 models, of which about 167,000 particles corresponding to full-length protein with high-resolution features were selected to generate two-dimensional class averages. Cryo-EM samples of DspE showed a severe orientation bias of the particles, which prevented high-resolution reconstruction of the cryo-EM density maps.
Cloning and in vitro transcription of avrE and dspE for oocyte experiments
The avrE or dspE open reading frame (ORF) was amplified with the following primer sets—avrE forward primer: 5′-TTGCCCGGGCGCCACCATGCAGTCACCATCGATCCACCGGA-3′ (Kozak sequence underlined); avrE reverse primer: 5′-CCTCTAGATTAGCTCTTCAGTTCGAACCCCTCT-3′; dspE forward primer: 5′-TTGCCCGGGCGCCACCATGGAATTAAAATCACTGGGAACTG-3′ (Kozak sequence underlined); dspE reverse primer: 5′-CCTCTAGATTAGCTCTTCATTTCCAGCCCTTCC-3′.
PCR-amplified avrE or dspE ORF (SrfI–XbaI fragment) was cloned into pGH19 (ref. 42) (digested with XmaI and XbaI) to create pGH-avrE or pGH-dspE. To prepare cRNA for oocyte injection, pGH-avrE or pGH-dspE was linearized with NheI, followed by in vitro transcription with T7 polymerase mMESSAGE mMACHINE Kit (Ambion).
For mutational analysis of DspE, point or deletion mutants of pGH-dspE were obtained using the Q5 Site-Directed Mutagenesis Kit (New England Biolabs) with the following primer sets—pGH-dspEΔβ-barrel (that is, ∆1278–1566 + ∆1649–1813), ∆1278–1566 forward primer: 5′-GCGGAGCCGGTGACCAGCAACGATA-3′; ∆1278–1566 reverse primer: 5′-ACTGTCCAGGGACAACAGCGTATTC-3′; ∆1649–1813 forward primer: 5′-GGAATAGCTCAGGCTAATCCGCAGG-3′; ∆1649–1813 reverse primer: 5′-GCTGTGGCTGTCCGCAGCCTGTTGA-3′; pGH-dspEK1399E/K1401E, K1399E + K1401E forward primer: 5′-CTGGAGTTTGAGCTGACAGAGGATGAG-3′ (underline indicates mutation point); K1399E + K1401E reverse primer: 5′-GCTGTTTTGTAGCGTTCCTTGCAGGGT-3′; pGH-dspEL1776E/L1777E/L1778E, L1776E + L1777E + L1778E forward primer: 5′-GAGGAAGAGGGGACGAGCAACAGCCTG-3′ (underline indicates mutation point); L1776E + L1777E + L1778E reverse primer: 5′-CGCTGGGGTATTGAAGCCTTCGCTTTT-3′.
Xenopus laevis oocyte preparation, injection and expression of DspE and AvrE
Oocytes were purchased as ovary from Xenopus1. The ovary was treated with 0.55 mg ml−1 collagenase B (0.191 U mg−1) in calcium-free ND96 saline43 (96 mM NaCl, 2 mM KCl, 1 mM MgCl2, 5 mM HEPES and 2.5 mM Na pyruvate, pH 7.5) for 20 min while on a nutating mixer at 21–22 °C. Immediately after treatment, the enzymatic solution was rinsed off the ovary with ND96 bath saline (96 mM NaCl, 2 mM KCl, 1 mM MgCl2, 1.8 mM CaCl2, 5 mM HEPES, 2.5 mM Na pyruvate and 0.5 mM theophylline, pH 7.5) several times, and cell clusters were spread on several 70-mm plastic culture dishes with ND96 bath saline for temporary storage in an 18 °C incubator. On the same day, the follicular cells and follicular membrane covering mature oocytes (stages IV and V) were manually peeled off with fine forceps, and oocytes were kept in ND96 ...
Comments
Post a Comment